3 General properties
3.1 Composition
3.1.1 Composition of Egyptian blue
EB is mainly constituted by cuprorivaite and it commonly contains some other minor components mainly due to incomplete reaction, unbalanced reagents, additives (i.e., fuxes, see Sect. 4.2), impurities from the reactants, and contamination from the crucibles or the production technique utilized. Furthermore, although EB is a very stable material, some compounds within it can also be the results of deterioration processes, e.g., due to overheating during the production (e.g., cristobalite or tridymite) or severe and prolonged harsh conditions as reported, i.e., in some archaeological fndings (see also Sects. 3.4 and 3.3.11).
In EB produced through the traditional melt-fux synthesis (see Sect. 3.3.4), the impurities are often concentrated in a glassy matrix surrounding practically pure cuprorivaite crystals (Delamare 1997). However, the occurrence of minor amounts of other crystalline phases is common, i.e., oxides or silicates (Tite et al. 1984; Bouherour et al. 2001; Nicola et al. 2019; Seymour et al. 2020). Overall the presence of excess silica (10–30%) above that necessary to produce cuprorivaite has been commonly reported in archaeological specimens as well as a slight excess of Ca or Cu, associated with the presence of CaSiO3 (wollastonite) or black copper oxide CuO (tenorite), respectively (Jaksch et al. 1983; Tite et al. 1984; Pagès-Camagna et al. 1999; Hatton et al. 2008; Fontana et al. 2020). The composition of the glassy matrix consists mainly of Si from excess silica, Ca and Cu from unreacted reagents, and Na and K from the fuxing agent (see Sect. 4.2.3). Al, Mg, and Fe, and to a minor extent Mn, Cr, and Ti are common impurities (Hatton et al. 2008; Lazzarini and Verità 2015; Baraldi et al. 2017; Fontana et al. 2020). Along with some Na and K, they could likely derive from silicate impurities (e.g., feldspar) present in the silica source (Hatton et al. 2008), or from the terracotta crucibles traditionally used for the production process (Mazzocchin et al. 2004; Warner 2011; Grifa et al. 2016; Nicola et al. 2019). Cl, S, and P impurities can derive from specifc contaminants in the reactants (e.g., chlorides, sulfates, phosphates, etc.) and are generally considered to be mainly associated with fuxes (Jaksch et al. 1983; Giménez et al. 2017; Dariz and Schmid 2022) but their presence can be as well due to other features, e.g., S from Cu2S ores used as a source of copper (Dariz and Schmid 2021).
The presence of As, Sn, Pb (El Goresy et al. 1998), and Zn (Ingo et al. 2013; Nicola et al. 2019) in the glass matrix or as oxides and silicates have been correlated with the copper source (i.e., copper alloys). The presence of Pb and Zn has been thought to be possibly also intentional and has been correlated with their function as fuxes (Nicola et al. 2019; Rodler and Kostomitsopoulou Marketou 2022). Traces of Co, Pt, Ni, Mg, Sr, Ba, Sb, Ag, Rb, Y, Zr, Nb, Sc, V, La, Ce, and C have also been considered in archaeological specimens (Jaksch et al. 1983; Tite et al. 1984; Riederer 1997; Ingo et al. 2013; Clegg 2014; Grifa et al. 2016; Zaina et al. 2019; Oudbashi and Hessari 2020). For example, the sporadic presence of a minor amount of Co has been investigated by Tite et al. to assess a possible intentional adding to improve the color of EB (Tite et al. 1984), while the content of Pt has been taken into account by Zaina et al. to obtain information on the provenance of EB, since Pt presence might suggest that EB was produced and imported from Eastern Desert of Egypt or Nubia (Zaina et al. 2019). Indeed, in archaeological science, the characterization of impurities in EB is extensively used as a method to try to trace its provenance and production technique (Nicola et al. 2019; Fontana et al. 2020; Martín et al. 2020; Seymour et al. 2020; Coccato et al. 2021; Dariz and Schmid 2021, 2022). For this purpose also isotopic techniques have been used on lead impurities and also on copper (Rodler et al. 2017, 2021).
3.1.2 Composition of Chinese blue and Chinese purple
EB has been by far more studied in comparison to its Chi- nese counterpart CB and the related CP. It could be roughly argued that archaeological CB and CP are quite similar to EB except for the fact that, instead of CaCuSi4O10 (cuprori- vaite), their main constituents are, respectively, BaCuSi4O10 (effenbergerite) and BaCuSi2O6 (colinowensite).
However, the system BaO–CuO–SiO2 is much more complex than the
CaO–CuO–SiO2 one (Wiedemann and Berke 2001), and thus some other differences are present between the average composition of EB and that of CB and CP. The main differ- ence is that at least four different stoichiometric barium–cop- per silicates can form during the production of CB and CP. Besides BaCuSi4O10 and BaCuSi2O6, they are BaCu2Si2O7 and Ba2CuSi2O7.
- BaCu2Si2O7 has been named Chinese dark blue (Berke et al. 2009). It is a dull blue compound corresponding to the mineral scottyite. It has been first identified in nature only recently, analogously to effenbergerite, col- inowensite, and wesselsite in the Wessels mine, Kalahari Manganese Field, South Africa (Yang et al. 2013). It has been synthesized with modern hydrothermal synthesis to be used as a pigment (Chen et al. 2014b; Rendón- Angeles et al. 2021) and has been extensively studied as an intriguing example of a spin S = 1/2 quasi-one-dimen- sional antiferromagnet (Tsukada et al. 1999; Glazkov et al. 2005, 2011).
- Ba2CuSi2O7 has been seldomly referred to as Chinese light blue (Ma et al. 2016). It is a whitish compound that has not yet been found as a natural mineral. It exists generally in two slightly different forms, i.e., α- and β-Ba2CuSi2O7 differing in the solid state structure just in the rotational orientation of the disilicate anions (Du et al. 2003; Berke et al. 2009). Only β-Ba2CuSi2O7 can be produced via solid-state synthesis, while α-Ba2CuSi2O7 can be produced only via hydrothermal synthesis (Du et al. 2003). β-Ba2CuSi2O7 is produced at around 600 °C and seems to show a higher thermal sensitivity in com- parison to the other three barium–copper silicates.
BaCu2Si2O7 and Ba2CuSi2O7 similar to cuprorivaite (EB) and related two-dimensional silicates have a layered structure but unlike them they are sorosilicates, i.e., less condensed silicates characterized by the presence of iso- lated double tetrahedral groups Si2O76− (Berke et al. 2009) (see also the following 3.2). For this reason, BaCu2Si2O7 and Ba2CuSi2O7 will not be a focus of this work, and will not be further detailed. However, it has to be noted that BaCu2Si2O7 has been occasionally found along with CB and CP in archaeological specimens (Berke et al. 2009; Xia et al. 2014; Ma et al. 2016; Zhang et al. 2019), while Ba2CuSi2O7 has not yet been identified in any archaeological findings (Lin 2018) and it is unlikely that has ever been historically used as a pigment due to its poor color features and the dif- ficulties in its preparation (Berke et al. 2009).
Other important features that differentiate CB and CP from EB are the following:
- other barium silicates can be present within CB and CP, e.g., BaSiO3 and Ba4Si6O16 (Sheptyakov et al. 2012; Chen et al. 2014a). They can form also from impurities, e.g., BaSnSi3O9 (Zhang et al. 2019)
- Pb has been historically largely preferred as fluxing agent for CP and CB (Bouherour et al. 2001; Li et al. 2015a; Zhang et al. 2019)
- BaSO4 is sometimes present and has been associated with the source of Ba (Li et al. 2015b) red Cu2O can be present (especially in CP) and has been considered responsible for a possible shift in the color of the pigments toward purple or red (Berke 2007) (see also Sect. 3.3.8).
3.1.3 Other 2D silicates related to Egyptian blue
The other EB-related 2D silicates of the gillespite group (i.e., wesselsite, gillespite, MCrSi4O10 (M=Ca, Sr, or Ba), and BaMgSi4O10) have only recently been artifcially produced and have been obtained in fairly pure form, with minor impurities only due to the specifc synthetic route employed. For further details, it is suggested to consult the specifc sources in the literature (Belsky et al. 1984; Miletich et al. 1997; Inoue et al. 2009; Bloise 2018; Zhong et al. 2020).
3.2 Structure
3.2.1 Cuprorivaite and other MCuSi4O10 (M=Ca, Sr, Ba)
Cuprorivaite (CaCuSi4O10) and the other two alkaline-earth copper tetrasilicates (i.e., efenbergerite or BaCuSi4O10 and wesselsite or SrCuSi4O10) are phyllosilicates that belong to the gillespite-group and crystallize in the tetragonal system, space group P4/ncc, Z=4 (Gaines et al. 1997; Kendrick et al. 2007).
Their structure was frst established by single-crystal X-Ray Difraction in 1959 (Pabst 1959) and subsequently reexamined and refned several times (Janczak and Kubiak 1992a; Bensch and Schur 1995; Mirti et al. 1995; Kendrick et al. 2007; Burzo 2009; Barbar et al. 2017) using also Rietveld refnement of powder neutron difraction (Chakoumakos et al. 1993; Hughes et al. 1997; Knight et al. 2010), Cu K-edge XAFS, EXAFS, and XANES (Hughes et al. 1997; Pagès-Camagna et al. 2006), Electron Paramagnetic Resonance (EPR) (Ford and Hitchman 1979; Mirti et al. 1995; Orsega et al. 2006; Binet et al. 2021) and high-resolution magic angle spinning (MAS) 29Si Nuclear Magnetic Resonance (NMR) (Stebbins 2017). Vibrational
Fig. 2 Schematic sketch of the condensation process of a four-membered silicate ring (Si40128−) to build up a Si40104− layer and its puckering induced by coordination of Cu2+ and M2+ (M=Ca, Sr, Ba). Adapted from Bouherour et al. (2001)
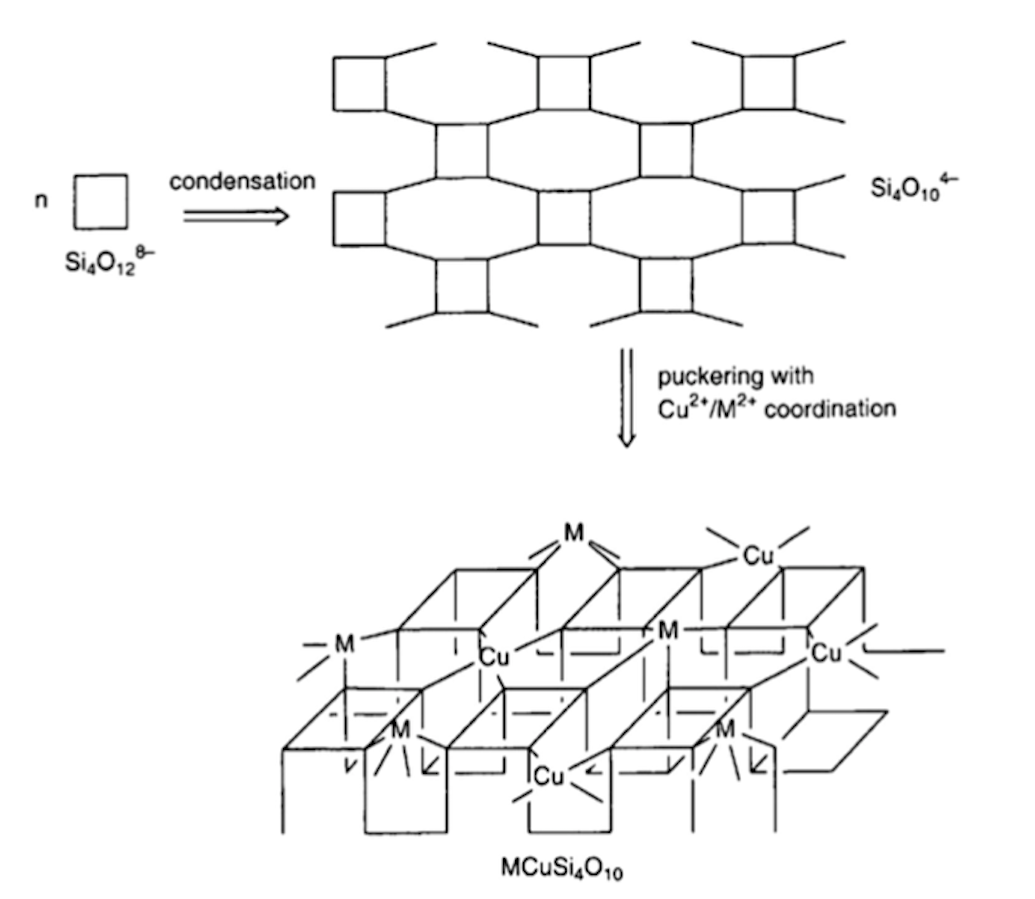
Fig. 3 Crystal structure of MCuSi4O10. In blue are shown Cu2+ ions, in gray the silicate tetrahedra. Reproduced with permission from Sgamellotti and Anselmi (2022). Original source: chemtube3d.com
and spectroscopic properties have also been studied, mainly using UV–Vis, FT-IR, and Raman spectroscopies (Mirti et al. 1995; McKeown and Bell 1997; Bruni et al. 1999; Baraldi et al. 2001a, 2006; Bouherour et al. 2001; Edreira et al. 2003; Ma et al. 2006; Pagès-Camagna et al. 2006; Barbar et al. 2017; Seymour et al. 2020). In this regard, it may be worth noting that spectroscopic details about EB, CB, and CP bulk commercial pigments (Kremer Pigmente) can be found in public databases, such as the CCR Pictorial Material Database (i.e., FORS spectra in the range 350–2200 nm, ATR–FTIR, and XRF) (Cavaleri et al. 2017), the FTIR and
Raman IRUG Spectral Database (irug.org), and the FORS Spectral Database of Historical Pigments in Diferent Binders (Cosentino 2014). The layered structure of cuprorivaite, efenbergerite, and wesselsite is sketched in Figs. 2, 3, and 4. It is built up of analogous nanosheets each constituted by a virtually endless layout of four and eight-membered rings of corner-shared SiO4 tetrahedra (Berke 2007). Each SiO4 tetrahedron is connected to two neighboring tetrahedra in the same square ring through two identical bridging oxygens O2 and to a third tetrahedron belonging to a diferent square ring through a diferent bridging oxygen O1 (Knight and Henderson 2007; Warner 2011). The fourth oxygen is a non-bridging oxygen anion O3 (Knight and Henderson 2007). Within each square ring, the four apical anions O3 all point in the same direction. Each square ring of tetrahedra is connected through the four O1 to four other identical square rings whose apical oxygen anions O3 all point in the opposite direction (Warner 2011). The nanosheet of tetrahedra is puckered by Cu2+ ions that form CuO4 groups by linking four oxygen anions O3 pointing in the same direction and belonging to four diferent (and thus non-directly adjacent) square-rings (Berke et al. 2009; Warner 2011). The Cu–O bonds are all equal in length (i.e., about 1.91 Å) and Cu2+ in the CuO4 groups are in an almost perfect D4h square planar geometry of coordination due both to lattice constraints and the Jahn–Teller efect (Mirti et al. 1995; Hughes et al. 1997; Kendrick et al. 2007; Berke et al. 2009; Knight et al. 2010; Sgamellotti and Anselmi 2022). Indeed, the stability of Cu2+ in square planar coordination is favored by the Jahn–Teller efect, since for some specifc electronic confgurations (and especially the d9 in Cu2+), the square planar symmetry often turns to be the preferred
Fig. 4 Projections of: a one-half of the cell (outlined) thickness viewed along [001] and b axiometric view with c vertical. The shaded tetrahedra represent SiO4 units, the large circles, alkaline-earth ions, and the small circles, Cu atoms. Reproduced with permission from Chakoumakos et al. (1993)
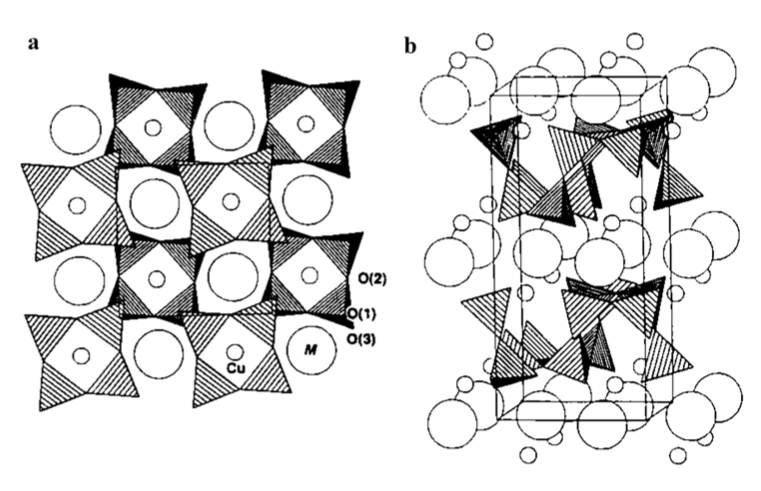
one in comparison with the elongated tetragonal (Td) coordination (Huheey 1978). The square-planar coordination descends as an extreme case of the progressive distancing of negatively charged ligands orthogonal to the square plane and their ultimate removal, as is the case of the oxygen coordinating Cu2+ ion in MCuSi4O10 (M=Ca, Sr, Ba) (Warner 2011). Indeed, in this case, there are no anions of any sort on the normal of the CuO4 square groups, and this might be considered an ideal case (Pabst 1959). Due to the puckering produced by Cu2+, each [CuSi4O10] 2− nanosheet results in a virtually infnite double layer of square rings of SiO4 tetrahedra. The two layers forming each nanosheet are internally strongly connected via the bridging oxygens O1. It has to be noted that the square rings of SiO4 tetrahedra and the CuO4 groups display specifc rotations and tilting within the double layer (Chakoumakos et al. 1993; Knight et al. 2010). The nanosheets [CuSi4O10] 2− are joined together by alkaline earth M2+ (M=Ca, Sr, or Ba) in a distorted cubic geometry (Knight and Henderson 2007). The M2+ ions are in eightfold coordination with four coordination sites in each neighboring nanosheet. Two of them are oxygen anions O3 also coordinated to Cu2+ in diferent CuO4 groups, while the other two are O2 bridging SiO4 tetrahedra belonging to distinct square rings (Giester and Rieck 1994; Knight and Henderson 2007; Warner 2011). The distance between two neighboring nanosheets depends largely on the dimension of the M2+ ions. It increases in the series Ca2+, Sr2+, and Ba2+ reaching a maximum of about 4.5 Å for Ba2+ (Burzo 2009). The shortest Cu2+–Cu2+ distance is between adjacent layers and is about 5.73 Å for cuprorivaite and 6.08 Å for efenbergerite. Within a layer, each Cu2+ ion has, instead, 8 neighbors at 7.25–7.30 Å (Binet et al. 2021). Despite their high concentration, Cu2+ ions are weakly antiferromagnetically coupled and can be roughly considered independent Cu2+ ions (Warner 2011; Binet et al. 2021). The weak coupling occurs within a single [CuSi4O10] 2− nanosheet, since the anion sublattice transmitting the super-exchange interactions is disrupted between the neighboring nanosheets (Binet et al. 2021).
The type of M2+ (i.e., Ca2+, Sr2+, or Ba2+) produces only minor variations in the double layer arrangement of SiO4 tetrahedra within the [CuSi4O10] 2− nanosheets (Miletich et al. 1997; Kendrick et al. 2007). However, Knight et al. point out that a slight increase in the magnitude of rotation and tilting of tetrahedra and in the rotation of CuO4 groups occurs to accommodate the larger and more electropositive Ba2+ within the crystal structure (Knight et al. 2010). According to Hughes et al. (1997), the refined characteristic unit cell parameters for the three alkalineearth copper tetrasilicates are the following:cuprorivaite a = b = 7.30128(4) Å and c = 15.12370(15) Åwesselsite a=b=7.36964(3) Å and c=15.57986(9) Åefenbergerite a=b=7.44534(6) Å and c=16.13971(22) Å Small deviations from these values are reported by diferent authors (Janczak and Kubiak 1992a; Chakoumakos et al. 1993; Giester and Rieck 1994, 1996; Mirti et al. 1995; Kendrick et al. 2007; Knight et al. 2010; Masunaga et al. 2015). The parameters of mixed alkaline-earth systems have been studied by Kendrick et al. in M1 1−x M2 xCuSi4O10, where M1 , M2 =Ca, Sr and Ba. They report that it is possible to successfully form a solid solution across the whole series of x when M1 and M2 are both Ca2+and Sr2+ or else Sr2+and Ba2+ but it is not possible to produce a solid solution when Ca2+ and Ba2+ are chosen together as M1 and M2 . They explain this feature by assuming that the excessive diference in ionic radii between Ca2+ and Ba2+ prevents the occurrence of a solid solution and, instead, leads to the formation of the two distinct endmembers, i.e., cuprorivaite and efenbergerite (Kendrick et al. 2007). Knight and colleagues accurately studied the system Sr1-xBaxCuSi4O10 (Knight et al. 2010) accounting also for the minor structural variations occurring at cryogenic temperatures (Knight and Henderson 2007). Masunaga et al. point out that at least for efenbergerite, two minor phase transitions occur at low temperatures (i.e., 87 K and 103 K) slightly difering the lengths of the a and b lattice parameters, thus breaking the tetragonal symmetry (Masunaga et al. 2015).
3.2.2 Structure of gillespite and its other group members
The structure of gillespite (i.e., BaFeSi4O10) was first described by Pabst in 1943 (Pabst 1943) and was used by the same author as a model for the analogous structure of the three alkaline earth copper tetrasilicates described in the previous paragraph (Pabst 1959). The structure has been refned and studied several times and has been reviewed by Burzo (2009). Unlike the three alkaline earth copper tetrasilicates, gillespite undergo an intriguing reversible frst-order phase transition induced by an increase in applied pressure.
The transformation begins in the range of 1.2–2.6 GPa and has been frst described by Strens (Strens 1966). It is associated with a shift in color from red to blue and it is due to a change in the iron coordination from square-planar to fattened tetrahedral (Knight and Henderson 2007). Single crystal, high-pressure X-ray difraction measurements showed that the phase transformation is associated with a structural phase transition from the tetragonal space group P4/ncc to the orthorhombic space group P21212. The two distinct phases are called, respectively, Gillespite I (tetragonal) and Gillespite II (orthorhombic) (Hazen and Burnham 1974; Hazen and Finger 1983). Gillespite shows a rare pattern of isolated Fe2+ in square-planar coordination with a high-spin S=2 state (Schofeld et al. 1998; Pascualini et al. 2015). All the three alkaline earth chromium tetrasilicate MCrSi4O10 (M=Ca, Sr, Ba) have been synthesized, although they have not yet been found in nature (Belsky et al. 1984; Miletich et al. 1997). They have a structure completely analogous to that of the minerals of the gillespite-group with only minor variation in the unit cell parameters. Analogously to what happens in alkaline earth copper silicates, the type of M2+ (Ca2+, Sr2+, Ba2+) afects mainly the c lattice parameter.
The substitution of the Q2+ in the MQSi4O10 series (Q=Cu2+, Cr2+, Fe2+) leads, instead, to only small changes in cell unit, equally infuencing the a and b parameters, without any signifcant change in symmetry. Analogously to what happens for MCuSi4O10, also in MCrSi4O10 the relatively high stability of the compound is favored by the Jahn–Teller efect (Belsky et al. 1984; Miletich et al. 1997). The Cr2+ cation in MCrSi4O10 occupies a square-planar coordinated site unique in oxide crystal chemistry (Miletich et al. 1997). BaMgSi4O10 seems to be another gillespite-group member that has not yet been found as a natural mineral. It has been frst described by Toropov et al. to crystallize in the tetragonal space group P4/ncc and to be isostructural with gillespite (Toropov 1962). More recently, diferent authors synthesized and characterized it (Inoue et al. 2009; Yuan et al. 2012; Zhong et al. 2020). However, there is some debate about the exact structure of this compound relying on a diferent route of synthesis and some difractograms discrepancies (Inoue et al. 2009; Zhong et al. 2020). Zhong et al. point out that more work is needed to reveal the exact crystal structure of this compound that if confrmed should contain a peculiar and intriguing Mg2+ in roughly squareplanar coordination (Zhong et al. 2020).
3.2.3 Structure of BaCuSi2O6, i.e., colinowensite
The main constituent of CP, i.e., BaCuSi2O6 (colinowensite) also crystallizes in the tetragonal system but unlike the other 2D alkaline-earth copper silicate described so far, is not a member of the gillespite-group and is not a phyllosilicate. Indeed, it is a cyclosilicate (Rieck et al. 2015).
Its crystal structure has been at frst assigned to space group I 44 m2 (Z=4) (Finger et al. 1989; Janczak and Kubiak 1992b) but Sparta and Roth in 2004 proposed an improved model placing it in the space group I41/acd with Z=16 (a=10.009(2), c = 22.467(6) Å) (Sparta and Roth 2004). Their model has been confrmed by further studies (Chen et al. 2014b; Stern et al. 2014; Rieck et al. 2015). Sparta and Roth also described a frst-order phase transition at high temperature (610 K) when superstructure refections disappear and the structure model shifts to space group I4/mmm with Z=4. Another frst-order phase transition occurs at low temperature (∼ 107 K) and is associated with an incommensurately modulated orthorhombic structure with space group Ibam (Samulon et al. 2006; Sheptyakov et al. 2012; Chen et al. 2014b). Low-Temperature High-Resolution Solid-State (cryoMAS) NMR has been used to investigate this structure that results composed of two diferent kinds of CuO4–CuO4 dimers (Stern et al. 2014) (see below within this paragraph). The low-temperature phase transition can be avoided down to about 1.5 K stabilizing the structure of BaCuSi2O6 with a partial replacement of Ba with Sr in SrxBa1−xCuSi2O6 (x<0.3) (Puphal et al. 2016; van Well et al. 2016; Song et al. 2019). It may be worth noting that the existence of other compounds isostructural with CP, e.g., pure SrCuSi2O6 has so far not been reported (Puphal et al. 2016; Song et al. 2019). The non-existence of CaCuSi2O6 has been related to its instability (Berke and Wiedemann 2000; Bouherour et al. 2001). Compounds such as CaMgSi2O6 (diopside), CaFeSi2O6 (hedenbergite), CaCoSi2O6 (copside) and CaNiSi2O6 (niopside) exist but display pyroxene structure (Masse et al. 1999; Szubka et al. 2022). They, thus, are not 2D materials and, therefore, will not be a focus of this work. Interestingly, there appears to be no known mineral or compound that exhibits a similar structure to that of colinowensite. However, colinowensite shares some structural similarities with alkaline-earth copper tetrasilicates and especially with efenbergerite. Indeed, also BaCuSi2O6 has a layered structure built up by nanosheets intercalated by Ba2+ ions, and its nanosheets are composed of square rings of silica tetrahedra linked together by Cu2+ forming CuO4
Fig.6 Simplified energy level diagram of Cu2+ ion in tetragonally distorted octahedral crystal field environment in cu-prorivaite lattice. Reproduced with permission from Li et al. (2014)
groups in almost perfect square planar coordination (Rieck et al. 2015) and with almost identical Cu–O bond length, i.e., about 1.92 Å (Pozza et al. 2000). The main diference between the structure of BaCuSi2O6 and that of the other 2D alkaline earth copper silicates is that in BaCuSi2O6 the square rings of tetrahedra are isolated and they are connected only by the CuO4 groups. Berke et al. point out that the diference between BaCuSi2O6 and the structures of compounds in the gillespite-group can be viewed as the condensation of the square rings of tetrahedra in the latter one, as sketched also in Fig. 2 (Berke et al. 2009). In BaCuSi2O6, the lack of this condensation precludes the formation of a virtually infnite network of silica tetrahedra. Indeed, the isolated square rings [Si4O12] 8− need Cu2+ ions to constitute a virtually infnite nanosheet [Cu2Si4O12] 4−. CuO4 groups link together four non-bridging oxygen anions from four diferent square rings of silica tetrahedra. As represented in Fig. 5, CuO4 groups face directly two by two, forming, therefore, a nanosheet composed of single square rings of silica tetrahedra connected in each corner by two parallel CuO4 groups. The nanosheets are then joined together by Ba2+ in tenfold coordination. Within the layer, at room temperature the distance between two opposite Cu2+ is quite small, i.e., 2.73–2.75 Å (Chen et al. 2014b; Stern et al. 2014; Rieck et al. 2015), which is close to the Cu–Cu distance in nanometer scale metallic clusters, i.e., 2.55–2.70 Å (Bazin and Rehr 2003). Actually, the Cu2+ ions (S=1/2) form a square lattice of CuO4–CuO4 dimers parallel to the c axis with antiferromagnetic coupling. Each dimer is roughly isolated from the others being the interdimer distance ∼ 7 Å in the same layers and ∼ 5.75 Å between dimers in adjacent layers (Sheptyakov et al. 2012; Stern et al. 2014). This unique feature has made BaCuSi2O6 a choice material to study Bose–Einstein condensation (BEC) of magnons in high magnetic felds and to study quasi-two dimensional isolated spin dimer
Fig. 7 Absorption spectra of cu-prorivaite at room temperature. Reproduced with permission from Li et al. (2014)
systems (Jaime et al. 2004; Sebastian et al. 2006a, 2006b; Harrison et al. 2006; Batista et al. 2007; Krämer et al. 2007; Rösch and Vojta 2007; Chen et al. 2014a; Mazurenko et al. 2014; van Well et al. 2016; Allenspach et al. 2020, 2021). BaCuSi2O6 has also been used as a model to study the behavior of isolated square rings of silica tetrahedra (McKeown and Bell 1997, 1998).
3.3 Color
3.3.1 Color of cuprorivaite and related 2D‑silicates
The three alkaline earth copper silicates owe their vivid blue color to their chromophores, i.e., Cu2+ ions in square-planar coordination, and the efect of the internal electric feld created by the entire crystal on them (García-Fernández et al. 2015, 2016). (Stern et al. 2014)
The geometry around Cu2+ ions induces the descent of symmetry from Oh to D4h, thus resolving the degeneracy within the Eg and T2g orbitals that resulted from the splitting of the octahedral crystal feld. In this way, four energy levels are formed (see also Fig. 6). A further minor splitting is due to spin–orbit interactions (Li et al. 2014). Three fundamental broad absorption bands associated with d–d transition thus exist, two of them in the visible and one in the NIR. The overall blue color of the three alkaline earth copper silicate is basically a consequence of the two absorption broad bands in the visible which are centered in the red and the green regions, while no absorption takes place in the blue region (see Fig. 7). In cuprorivaite, according to Li et al., the absorption bands are, respectively, in green at 536 nm (2 B1g—>2 A1g), in red at 630 nm (2 B1g— > 2 Eg), and in NIR at 764 nm ( 2 B1g— > 2 B2g) (Li et al. 2014). Slight differences are reported by other authors, especially for the NIR band that is often reported at a little longer wavelength, i.e., around 780 nm (Pagès-Camagna et al. 2006; Accorsi et al. 2009; Warner 2011; Borisov et al. 2013; García-Fernández et al. 2015; King et al. 2016; Binet et al. 2021).
Fig. 8 Image of modern EB and related pigments marketed by Kremer Pigmente. From the left: 10,064 Egyptian green, 100,601 Egyptian blue (particles<to 10 μm), 10,060 Egyptian blue (particles<120 μm), 10,072 Han blue (i.e., CB) and 10,075 Han purple (i.e., CP) (colour fgure online). Adapted from Nicola (2019)
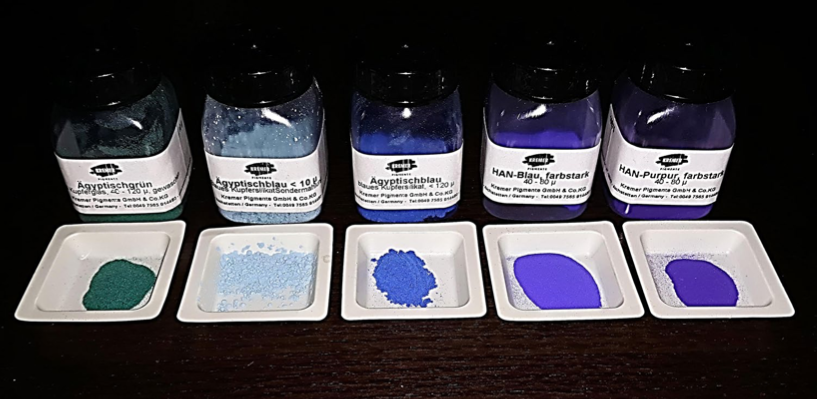
Only minor diferences are reported to occur among the three MCuSi4O10 species (Kendrick et al. 2007). However, Borisov et al. point out that on going from CaCuSi4O10 to BaCuSi4O10 a shift is noticeable (∼36 nm) in the NIR absorption band associated with 2 B1g—>2 B2g, although in the visible region just a limited bathochromic shift (∼10 nm) of the 2 B1g—>2 A1g band is observed (Borisov et al. 2013). Kendrik et al. report some slight shift in color properties in the M1 1−x M2 xCuSi4O10 series, where M1 , M2 =Ca and Sr or else Sr and Ba. Overall, they point out that only a very limited whitening takes place with the replacement of Ca2+ by Sr2+, while a more appreciable efect is associated with the replacement of Sr2+ by Ba2+ (Kendrick et al. 2007). May be worth noting that the three MCuSi4O10 as well as the other MQSi4O10 (M=Ca, Sr or Ba and Q=Cu, Fe, Cr and possibly Mg) and also BaCuSi2O6 all show dichroism, i.e., they show two distinct colors when they are observed in different orientation under polarized light (Belsky et al. 1984; Miletich et al. 1997; Schofeld et al. 1998; Wiedemann and Berke 2001; Eastaugh et al. 2004; Warner 2011; JohnsonMcdaniel et al. 2012; Skovmøller et al. 2016). In cuprorivaite, e.g., the ordinary ray, ω is deep blue, and the extraordinary ray, ε is pale pink (Fouqué 1889; Warner 2011). The overall color of the other members in the gillespite group MQSi4O10 is also due to the Q2+ atom in roughly square-planar coordination that acts as a chromophore and to lattice constraints. MCrSi4O10 has tones in the range of pink, magenta, and salmon-red (Belsky et al. 1984; Miletich et al. 1997). It has three broadband absorptions that show some resemblance with those of MCuSi4O10 but seem blueshifted. In CaCrSi4O10 the most intense absorption reaches a maximum at 511 nm for light polarized in (001).
For light polarized parallel to the c-axis, an absorption maximum occurs at 453 nm. A broad, weak absorption also occurs centered at 670 nm in (001) (Belsky et al. 1984). Gillespite is normally red but, unlike the other members of the group, it changes its color at high pressure, turning blue as a consequence of its phase transition (see Sect. 3.2.2). BaMgSi4O10 is white (Zhong et al. 2020) due to the absence of any possible d–d transition. Like other alkaline-earth copper silicates, BaCuSi2O6 also owes its color to Cu2+ in square-planar coordination but García-Fernández et al. point out that a signifcative diference exists in comparison to MCuSi4O10 in the contribution from the internal electric feld created by the crystal (GarcíaFernández et al. 2016). Berke et al. report that BaCuSi2O6 in its pure state (degree of purity 99.5%), obtained through melt-fux synthesis, seems to be not purple, but dark blue. A purple shade in BaCuSi2O6 has been suggested to be the result of the formation of impurities of red Cu2O (cuprite), as a consequence of the high temperature reached in the production process (Berke 2007). However, Chen et al. point out that BaCuSi2O6 produced through hydrothermal synthesis is markedly purple even when no Cu2O phase is detected through XRD analysis (Chen et al. 2014b). The exact color of BaCuSi2O6 specimens seems, indeed, to be related to the dimension of the crystals (Wiedemann and Berke 2001). In fact, also BaCuSi2O6 found as natural colinowensite appears dark blue when in large crystals, while it seems distinctively purple when crystals are smaller than 40 µm (Rieck et al. 2015).
3.3.2 Egyptian blue, Chinese blue, and Chinese purple
EB, CB, and CP pigments are not single phases of their main constituents and it has to be said that they owe a color that can take on diferent tones due to many factors. EB commonly ranges in color from ashy or pale blue to intense deep blue. Sometimes, it can also get blackish or greenish shades. When pure and in the same particle sizes, the color of CB is in general very similar to that of EB (Berke 2007). However, CB can often get a purplish shade, and less commonly than EB, it gets blackish or greenish. The tone of CP is generally purple but can also have shades of blue, pink, and red, and can get blackish. Some commercial EB, CB, and CP are shown in Fig. 8 also in comparison with Egyptian green, a vitreous material related to EB and better detailed in Sect. 4.1.1 The main features that can infuence the color of EB, CB, and CP are listed in the following paragraphs.
3.3.3 Efect of particle size
Coarse EB, CB, and CP are generally deep blue if constituted of clusters of large crystals (Augusti 1967; Tite et al. 1987; Berke and Wiedemann 2000; Canti and Heathcote 2002). If ground to less than 15 μm EB gets quite pale but can stay deep blue if kept greater than 30 μm (Pagès-Camagna et al. 2010). The efect is evident in Fig. 8. The pale aspect of thin particles of EB and CB is generally due to the increase in the scattering of light (Kakoulli 2002). This seems true especially if the EB particles are the result of the grinding of pigments with high amounts of glass matrix. The pigments produced with a limited amount of glass matrix or via sol–gel, seem less afected by the whitening due to grinding (Chopin and Macaudiere 1994). However, pigments with a low amount of glass matrix are in general constituted of fne-textured agglomerate of particles, therefore, of relatively light tones, but, especially the sol–gels ones, can be produced in very thin particles of few μm or less without losing their quite brilliant blue tone (Chopin and Macaudiere 1994). The possibility of producing EBs made up of particles of diferent sizes has historically been widely exploited to obtain a series of pigments with diferent tones of blue as suggested by Theophrastus (Eichholz 1965; Katsaros et al. 2010; Kostomitsopoulou Marketou et al. 2020). CP is diferent from EB and CB in the fact that the crushing of large blue crystals of CP does not produce a simple whitening but turns it to a purplish color as does its streak (Rieck et al. 2015). May be worth noting that the exfoliation in nanosheets of cuprorivaite (i.e., EB), efenbergerite (i.e., CB), and wesselsite generally induces an ashy tone (Johnson-McDaniel and Salguero 2014) that has been related to their dichroic properties and the reversible weakening of the 536 nm (2 B1g—>2 A1g) absorption in the green (Johnson-Mcdaniel et al. 2012).
3.3.4 Efect of the amount of fux and the route of synthesis
The traditional way to produce EB, CB, and CP consists of a high-temperature synthesis involving a fux that promotes the melting of silica in a liquid phase of silicates and the formation of crystals within it through nucleation and growth (see also Sect. 4.2).
For this reason, it is often referred to as melt-fux synthesis or salt-fux synthesis (Warner 2011; Johnson-McDaniel and Salguero 2014). If the amount of
Fig. 9 SEM–EDS images showing cross sections of EB particles included in resin: A melt-fux synthesis and B solid-state synthesis. Reproduced with permission from Nicola et al. (2019)
Fig. 10 Images acquired through optical microscopy of powders of diferent EB pigment modernly synthetized. The powders have been embedded in polyester resin and lapped. KR is from Kremer Pigmente; ZA4 is obtained through high-temperature melt-fux synthesis using zinc as a secondary fux; ZM4 is analogous to ZA4 containing excess of Ca, Zn and silica; ZE4 is obtained with conventional solidstate synthesis (i.e., without any fux), and ZR4 is analogous to ZE4 but contain unreacted reagents due to diferent raw materials used. Reproduced with permission from Nicola et al. (2019)
fux is enough (e.g., above 2% w/w in the fnal product), then the crystals of cuprorivaite, efenbergerite, or colinowensite will end up being embedded into quite an extensive silicate glass matrix (Tite et al. 1987; Delamare 1997; Wiedemann and Berke 2001). An example of EB obtained via melt-fux synthesis is shown in Fig. 9A. The glass matrix contains copper and has its own color that in general ranges between blue and green, and takes part in the overall color of the pigments (Onoratini et al. 1987; Etcheverry et al. 2001;Pagès-Camagna et al. 2006). Melt-fux synthesis generally produces the quick growth of large crystals of MCuSi4O10 (Delamare 1997; Johnson-McDaniel and Salguero 2014) and, therefore, gives the pigments an overall intense and deep color (Ullrich 1979; Wiedemann and Bayer 1982). This feature is evident in Fig. 10, where ZA4 is a sample of EB produced via high-temperature melt-fux synthesis with zinc as secondary fux and KR is a sample of commercial EB, arguably obtained by conventional melt-fux synthesis using sodium or potassium compounds as fuxing agents. However, if the amount of glass formed is very large and there is little copper within it, or if some colorless glass has been deliberately added in certain steps of the production, then the EB obtained will be diluted and the color will be pale blue even for solid masses of EB obtained via melt-fux synthesis (Jaksch et al. 1983; Lee and Quirke 2000). If the fux present in the high-temperature synthesis is close to zero then the glass phase will be limited or almost absent (Pradell et al. 2006). In this case, the temperature required for an efcient synthesis will be higher, e.g., 975 °C instead of 875 °C (Johnson-McDaniel and Salguero 2014), the synthesis will be possibly governed mainly by difusion laws (Delamare 1997), and will be in general defned as solid-state synthesis (Johnson-McDaniel and Salguero 2014). However, Pradell et al. point out that EB crystals are formed through nucleation and growth within a liquid phase, even for fux content as low as 0.3 wt%, i.e., arguably in any ancient EB (Pradell et al. 2006). As shown in Fig. 9B, the crystals of cuprorivaite formed by solid-state synthesis (i.e., without the adding of any fux) are not embedded in a glass matrix and are smaller, producing an overall lighter color of the pigment (Delamare 1997; Nicola et al. 2019). In fact, they consist of fne-textured crystals in small clusters uniformly interspersed on the surface of unreacted quartz grains (Tite et al. 1987; Nicola et al. 2019). ZE4 in Fig. 10 is an example of EB produced without any fux, i.e., via solid-state synthesis. It is evident its lighter color if compared with pigments produced via melt-fux synthesis (e.g., ZA4 and KR). When hydrothermal synthesis is used to produce crystals of cuprorivaite, efenbergerite, and wesselsite they have been reported to be bright blue (Chen et al. 2014a; JohnsonMcDaniel et al. 2015; Zhang et al. 2018), while those of colinowensite looks purple (Chen et al. 2014b). Solution combustion synthesis has been reported to produce EB pigments with a color that depends on the preheating temperature and that ranges between midnight blue (700 °C), teal (750 °C), dark cyan (800 °C), and dark turquoise (850 °C) (Panagopoulou et al. 2016). Sol–gel syntheses of alkaline earth copper silicates can produce pigments in a wide range of colors in blue and violet tones. It is claimed that such pigments are very easily ground in mild conditions reaching a much thinner grain size, without altering the color of the products unacceptably. They would also have excellent coloring power and excellent hiding power (Chopin and Macaudiere 1994).
3.3.5 Efect of non‑stoichiometric or unreacted reagents
The excess of copper or its incomplete reaction often leads to the formation of colored impurities (see, e.g., ZR4 in Fig. 10). In EB they are often present as black CuO (Johnson-McDaniel and Salguero 2014; Bloise et al. 2016).
CuO is a common occurrence when EB is produced with stoichiometric ratios of reagents, since in this case part of the silica in the mix of reagents cannot take part in the reaction that is forming cuprorivaite. In fact, some SiO2 reacts, e.g., with sodium from the fux to form the glass matrix or stays unreacted forming grains in the core of particles of cuprorivaite. The lack of silica leads to the formation of byproducts such as CuO and CaSiO3 instead of CaCuSi4O10. Arguably, this side efect has been historically countered using the excess of 10–30% SiO2 that has been reported in archaeological samples by almost any author (see Sect. 3.1.1). When CuO is present, it gives an overall gray-blackish unpleasant color to the pigment. CuO can be removed from EB by washing the product with a strong acid, e.g., HCl, in general with subsequent annealing of the pigment, which will turn to bright blue (Canti and Heathcote 2002; Moussa and Ali 2013; Johnson-McDaniel and Salguero 2014; Berdahl et al. 2018; Fontana et al. 2020). In the case of CB, a lack of silica during manufacturing can lead to the formation of a wider range of byproducts, since the BaO–CuO–SiO2 system is more complex than CaO–CuO–SiO2. Indeed, at least four stoichiometric ternary phases exist, i.e., BaCuSi4O10 (efenbergerite, blue),
BaCuSi2O6 (colinowensite, purple), BaCu2Si2O7 (scottyite, dark blue), and Ba2CuSi2O7 (light blue) (see also Sect. 3.1.2). Although only one of them is generally largely predominant, a mix of the frst two (or occasionally three) compounds generally occurs in CB and CP pigments obtained via melt-fux synthesis. The exact ratio infuences the specific color of each CB or CP pigment produced (Berke and Wiedemann 2000; Wiedemann and Berke 2001). A limited excess of silica seems not to impact heavily the overall color of EB and CB. On the contrary, during CP manufacturing, it can promote the formation of impurities Rendiconti Lincei. Scienze Fisiche e Naturali 1 3 constituted by CB, giving it a bluish tone. A slight excess of alkaline earth can produce some whitening due to the formation of barium or calcium compounds such as CaSiO3 (wollastonite) in EB. It may be worth noting that CaSiO3 is also produced from EB in presence of a high amount of fux and temperatures overall higher than its thermal stability. In this case, it is generally associated with a greenish copperbearing glass phase, see also Sects. 3.4.2 and 4.1.1 (PagèsCamagna and Colinart 2003; Hatton et al. 2008; Grifa et al. 2016; Kostomitsopoulou Marketou et al. 2020).
3.3.6 Efect of the raw materials used
The synthesis of EB, CB, and CP can be accomplished with a wide variety of reagents. Many diferent sources of silica, copper, and alkaline earth can be exploited as well as other additives commonly used, i.e., fuxes. In general, pure metals or alloys, oxides, carbonates, nitrates, and (seldom) sulfates, sulfdes, and chlorides are the preferred ones (Riederer 1997; Qin et al. 2016; Zhang et al. 2016; Lin 2018; Nicola et al. 2019; Dariz and Schmid 2021; Kiss et al. 2022).
However, the exact type of each reagent can promote the formation of specifc impurities in the fnal product or can infuence the dynamic of the reaction impacting thus the overall fnal color of the pigments. The large white particle in the bottomright corner of Fig. 9B and ZR4 in Fig. 10 show, e.g., how the use of pure copper metal powder as a source of copper could be not recommended in solid-state synthesis. Indeed, even if the copper powder is efective in melt-fux synthesis, it became detrimental if fux is not present, originating a lot of unreacted particles that will impact the general color (Nicola et al. 2019). The use of chlorides and sulfates (or sulfdes) can instead produce the formation of separate melt systems called galle (Rehren 2008; Dariz and Schmid 2022). Some efects on the color due to diferent reagents have been described, e.g., for the melt-fux synthesis of EB
(Tite et al. 1984; Bianchetti et al. 2000; Mazzocchin et al. 2004; Hatton et al. 2008; Bloise et al. 2016; Rodler and Kostomitsopoulou Marketou 2022) and for CB and CP (Berke and Wiedemann 2000; Wiedemann and Berke 2001; Corbiere 2009; Qin et al. 2016; Lin 2018). It has been reported that the chemical nature of the fux can infuence the tone of the blue (Warner 2011). It may be worth noting that the fnal result not only depends on the chemical nature of each reagent but is also infuenced by the size and shape of the grains, since, e.g., thin silica particles will result in a minor amount of unreacted reagents (Schippa and Torraca 1957; Ullrich 1979; Mazzocchin et al. 2004; Kakoulli 2009).
3.3.7 Efect of impurities
Some impurities in the reactants, e.g., NaCl (Giménez et al. 2017), Fe (Bianchetti et al. 2000), or a high amount of Zn and Ca (Nicola et al. 2019) can promote the formation of greenish byproducts in EB (see, e.g., ZM4 in Fig. 10). Limited amounts of As, Sn, Pb, and Zn have instead not been reported to alter signifcantly the color of EB even if more work on this topic is needed (Kakoulli 2002). However, some of them (i.e., Pb and Zn) can act as fuxing agents and have efects similar to those described in Sect. 3.3.4 Efect of the amount of fux and the route of synthesis (Nicola et al. 2019; Rodler and Kostomitsopoulou Marketou 2022). Kostomitsopoulou Marketou et al. extensively describe the efects of some common impurities on a large group of archaeological pellets of EB (Kostomitsopoulou Marketou et al. 2020, 2021). The chromatic efects of impurities in CB and CP have also been studied even if they are more difcult to describe, since the BaO–CuO–SiO2 system is more complex than CaO–CuO–SiO2. Some detailed discussions are however available in the literature (Wiedemann and Bayer 1997; Berke and Wiedemann 2000; Bouherour et al. 2001; Berke et al. 2009; Corbiere 2009; Lin 2018).
3.3.8 Efect of the temperature and length of production
If the temperature of the reaction in the traditional synthesis is too high, e.g., above 1050–1100 °C, cuprorivaite may start to decompose (Mazzocchin et al. 2004) turning EB into a greenish concoction containing wollastonite, high-temperature polymorphs of silica (i.e., tridymite and cristobalite), and a copper-bearing glassy phase (Grifa et al. 2016).
Furthermore, if a large amount of fux is present, then the process takes place more markedly and an extensive green glassy matrix is formed. These conditions have historically been used to deliberately produce a green pigment known as Egyptian green or green frit (Pagès-Camagna and Colinart 2003; Hatton et al. 2008; Grifa et al. 2016). A modernly produced commercial Egyptian green is shown in Fig. 8, for further details on it see also Sect. 4.1.1. In the synthesis of CB, analogous decomposing effects take place at a similar or possibly higher temperature, i.e., above 1200 °C (Wiedemann and Bayer 1997). CP is instead more temperature sensitive than EB. Wiedemann and Berke report that CP already at 1000 °C can decompose according to the general route: 3BaCuSi2O6 → BaCuSi4O10 + 2BaSiO3 + 2CuO turning thus partly in CB and getting blackish due to the formation of CuO. They also argued that if the temperature is above 1050 °C, CP can turn also reddish-purple as a consequence of the transformation of CuO (black) into Cu2O (red) and oxygen (Wiedemann and Berke 2001). CP melts with decomposition at around 1100 °C (Berke and Wiedemann 2000). Also in hydrothermal syntheses the temperature of
the reaction impact the color of the pigments produced (Johnson-McDaniel et al. 2015; Sun et al. 2021). In this case,the fnal color arguably depends also on other aspects, such as pH and concentrations of reactants (Chen et al. 2014a, b; Rendón-Angeles et al. 2021). The efects of temperature in solution combustion synthesis have been described in Sect. 3.3.4. Overall, the duration of the high-temperature production processes and the cooling rate can induce some changes in the fnal color of the pigments produced in each type of synthesis. In melt-fux synthesis, longer duration and slow cooling can promote, e.g., the formation of larger crystals of more intense color (Schippa and Torraca 1957; Riederer 1997; Warner 2011), while a short time of reaction and a fast cooling can lead to smaller crystals and thus to a paler color of the pigments. A short time of production can lead also to large amounts of unreacted reagents. May be worth noting that the span in the length of synthesis reported in the literature can be very wide, ranging from a few minutes (Pradell et al. 2006; Kiss et al. 2022) to more than 300 h (Schippa and Torraca 1957; Riederer 1997; Warner 2011).
3.3.9 Refractive Index and mixing with binding media
The presence of binding media is another factor that can modify the aspect of the pigments. Indeed, the hiding power and the saturation of pigments depend on the difference between their refractive index (RI) and that of the binder (Cosentino 2015). EB has refractive indices of 1.636 w (ordinary ray, electric feld perpendicular to the c-axis) and 1.591 e (extraordinary ray) (Berdahl et al. 2018; Sobik et al. 2021). CB has almost identical values (i.e., 1.633 w and 1.593 e), while CP has higher values of 1.72 and 1.74 (Eastaugh et al. 2004). The RI values of the pigments are quite diferent from the ones of many water-based binding media. For this reason, EB, CB, and CP pigments are in general light and opaque when used, e.g., with gum arabic, simply showing a slight efect of whitening due to dilution. Oils, waxes, and many polymeric resins have RIs closer to that of EB, CB, and CP. If they are used as binding media for the three pigments, they decrease the scattering efect of the powders and produce more intense and deep colors. However, they decrease also the hiding power of the pigments and increase transparency efects (Kakoulli 2002; Daniels et al. 2004). EB has been used to paint not only on walls or wood but also on terracotta. Osanna and Rescigno report that for this purpose lime is used as binding media (Osanna and Rescigno 2022) arguably leading to light blue tones.
3.3.10 Interaction with other pigments
EB, CB, and CP are generally considered to be compatible with any other pigment. The mixing with pigments (or any other extraneous material) can be used to modify their color. However, the careful exploitation of partial transparency allows the production of color efects even simply by superimposing thin layers of, e.g., EB on layers containing other pigments (Osanna and Rescigno 2022). Historically, EB has been used to produce green tones by mixing it with, e.g., orpiment or iron-oxide yellows (Scott 2016) or to improve the tone of green pigments by adding it, e.g., to green earth or Egyptian green (Mazzocchin et al. 2003; Perez-Rodriguez et al. 2015; Nicola et al. 2018b; Bracci et al. 2022). It was also used to obtain gray, brown, purple, ocher, and skin colors by adding, e.g., iron-oxides or cinnabar (i.e., vermilion) (Edreira et al. 2003; Aliatis et al. 2010; Fermo et al. 2013; Skovmøller et al. 2016; AIRPA 2021; Bracci et al. 2022; Osanna and Rescigno 2022). In general, EB has been lightened by mixing it with white pigments (Osanna and Rescigno 2022), such as calcium carbonate, calcareous clay (Augusti 1967), or lead white (Aliatis et al. 2010). Sometimes, EB has been added only in very small amounts, likely to optically achieve a perceived higher intensity of the white sensation (Edreira et al. 2003; Hallmann et al. 2021). Early uses of EB to improve the whiteness of white pigments predate Roman times. G. Chiari reported, e.g., it in paintings within the tomb of Tutankhamun (personal communication, December 3, 2016). CP and CB have also been mixed with other pigments, e.g., lapis lazuli blue in beads of the eighth century BCE (see Fig. 19). In polychromies of the Qin Shi Huang Terracotta Army, CP has been found mixed, e.g., with vermilion, azurite, and iron oxide (Herm et al. 1995; Bouherour et al. 2001; Berke 2007), while in a Western Han dynasty Terracotta Army, CP has been reported mixed with chalk (Wei et al. 2012). The possibility to add organic dyes to improve or modify the color of EB, CB and CP should not be ruled out. In this regard, it may be worth noting that, in Book XXXIII of Naturalis Historia, Pliny the Elder reports a practice consisting in boiling EB with plant juice to improve its color (Bostock and Riley 1857). However, no organic dyes have been reported so far in any archaeological specimen of EB, CB, or CP, likely due to their labile nature (Augusti 1967).
3.3.11 Efects of deterioration processes
In some circumstances also very stable pigments such as EB, CB and CP have been reported to sufer some decay that can impact their color (see also Sect. 3.4.3). More specifcally, a color alteration toward green has been reported in many EB of Egyptian origin (Schiegl et al. 1989, 1992; Abadir 2014; Giménez 2015a, b; Scott 2016; Moussa et al. 2021), while an example in EB of Roman times has been, e.g., reported in the coastal city of Formia, in a hypogeum environment with a very high relative humidity and high level of dissolved calcium carbonate and possibly calcium bicarbonate and chlorides (Nicola et al. 2006).
In general, such color alterations have been attributed to the copper chloride cancer mechanism (Schiegl et al. 1989, 1992; Abadir 2014; Giménez 2015a, b; Scott 2016; Moussa et al. 2021) and the consequent formation of green basic copper chlorides. However, there is some debate about basic copper chlorides found in EB of archaeological sources. The general consensus is that they generally are signs of degradation of sensitive pigments, such as azurite and malachite, and possibly even of more stable pigments, such as EB (Scott 2016; Blom-Böer and Warburton 2020; Hallmann et al. 2021). Nevertheless, it seems not possible to rule out that at least occasionally basic copper chlorides have been used as synthetically prepared green pigments (El Goresy et al. 1986; Hedegaard et al. 2019; Švarcová et al. 2021). It seems that it cannot be ruled out that chloride impurities in natron or other reactants used in the production of EB have a role in its decay. However, in some specifc experimental syntheses, Giménez et al. report that adding NaCl among the reactants does not induce the formation of copper chlorides in products, but rather produces a mixture with features similar to Egyptian green (see also Sect. 4.1.1) (Giménez et al. 2017). Another mechanism of decay that can turn EB, CB, and CP toward green (or brown) is the very common phenomenon of the yellowing of paints, binders, or coatings (Scott 2016). The blackening of painting layers containing EB is a common occurrence in many archaeological fndings and has been reported already in 1934 by Lucas (Lucas 1934, Lucas and Harris 1962 and Scott 2016). Daniels et al. concluded that it can be due to two main causes. The frst seems prevalent and is the darkening of the organic materials used as pictorial binders and varnishes. In this regard, it may be worth noting that possibly the natural photooxidation of many organic compounds could be intensifed by the presence of EB due to its catalytic efects (Coccato et al. 2017; Gao et al. 2022). The second hypothesis of Daniels et al. regarding the blackening of EB is that it can be due to the deposition of dirt followed by the formation of gypsum-rich black crusts over the rough surface of the coarse pigment (Daniels et al. 2004). The efect seems accentuated by the fact that EB has often been used coarser than the other pigments to preserve its deep blue tone (see Sect. 3.3.3). This produces thicker pictorial layers that require more binding media than those containing other pigments and that show a rough surface that can easily trap dust and deposits. The blackening of EB has been at frst argued to be linked to the formation of black CuO, maybe also mixed with other degradation products (Lucas 1934; Scott 2016).
Kendrick et al. point out a possible light sensitivity of cuprorivaite, wesselsite, and efenbergerite that could lead to the blackening of EB and CB due to the local formation of black CuO (Kendrick et al. 2007). However, Lee and Daniels et al. reported that no signifcant presence of CuO is detectable in EB from blackened areas, concluding that the blackening of EB in archaeological samples is not due to the presence of CuO (Green 2001; Daniels et al. 2004). For further details see also Sect. 3.4.5. It has to be noted that also other chromatic alterations can be commonly observed in many samples of EB of archaeological sources, e.g., toward brown, or dark green. They can be explained as a combination of some of the mechanisms already discussed (Scott 2016). CB has been reported to be much more stable than CP (Wiedemann and Bayer 1997) and even more stable than EB (Salguero et al. 2014). It is so stable that it can be argued that CP could get bluish and blackish over time due to the at least partial transformation into the more stable CB along with CuO (Wiedemann and Bayer 1997; Berke et al. 2009; Xia et al. 2014). CP fades rapidly and decomposes when exposed to diluted strong acids. Contact with aqueous oxalic acid can result in the formation of a turquoise-bluish residue. Since lichens excrete oxalates or even oxalic acid, this is a possible threat to the conservation of CP in archaeological sites (Wiedemann and Bayer 1997). It has been argued that for this reason, the light blue color of the trousers of some Terracotta Warriors may not be the original color, but rather an alteration of CP due to the presence of oxalic acid (Wiedemann and Berke 2001). Nevertheless, the conservation issues of CP and CB on archaeological fnds are overall not particularly a matter of concern in comparison to those of the other ancient blue pigments such as azurite or lapis lazuli which can easily fade even in humid or slightly acidic environments (Plesters 1966; Berke et al. 2010). The ancient organic artistic materials used to apply EB, CB, and CP are generally far more sensitive to color alteration or conservation issues than the pigments themself. For example, the greenish tone observed in the so-called blue room in Ariadne’s House in Pompei is due to the collapsing of the painting layer and not to EB alteration (Prieto-Taboada et al. 2021), while the darkening of many statues of the Terracotta Army of Emperor Qin Shi Huang has overall been due to the faking of the ground layer of Qi lacquer behind the polychromies and not to the alteration of CP (Blänsdorf and Xia 2006; Bonaduce et al. 2008).
3.4 Stability
3.4.1 Overview
Alkaline earth copper tetrasilicates are very stable over time as suggested by the very high number of archeological fndings of EB and CB produced thousands of years ago and in excellent conditions (Wiedemann and Bayer 1997; Li et al. 2014). CP is more sensitive than EB and CB (Berke et al. 2010). However, CP is still overall stable and is generally found sound in archaeological fndings (Wei et al. 2012).
3.4.2 Thermal stability
EB is extremely stable over a wide range of temperatures (Li et al. 2014) starting to decompose around 1050–1100 °C (Mazzocchin et al. 2004). CB seems to be even more stable, decomposing around 1200 °C (Wiedemann and Bayer 1997). The 2D materials related to EB and CB are arguably all stable from cryogenic temperatures to 1000 °C or more. Among the copper-containing members, CP is the most sensitive to high temperatures starting to decompose at about 1000 °C (Wiedemann and Berke 2001). For further details on thermal stability see also Sect. 3.3.8. May be worth noting that the specifc thermal stability of the pigments produced through traditional synthesis seems to be infuenced by the presence in the glass matrix of elements such as Na+ and K+ originating from fuxes. A high amount of fuxes, could arguably decrease the thermal stability of the pigments produced, and in any case can prevent their formation (Mazzocchin et al. 2004). The diferent amounts of fuxes in the specimens analyzed by diferent authors could possibly account for the discrepancies in the range of thermal stability reported in the literature and emphasized, e.g., by Jaksch et al. (1983), Mazzocchin et al. (2004) and Warner (2011).
3.4.3 Stability in the acid environment
Cuprorivaite, effenbergerite, and wesselsite have been reported and confrmed to be very resistant to acid attacks, even against concentrated, hot, and strong acids, such as HCl (Pabst 1959; Riederer 1997; Wiedemann and Bayer 1997; Berke and Wiedemann 2000; Johnson-McDaniel and Salguero 2014; Berdahl et al. 2018; Fontana et al. 2020). On the contrary, BaCuSi2O6 (i.e., colinowensite) and BaFeSi4O10 (i.e., gillespite) have been reported to be readily leached and/or decomposed when in contact with acids (Pabst 1958, 1959; Wiedemann and Bayer 1997)
It seems unknown whether MCrSi4O10 (M=Ca, Sr or Ba) and BaMgSi4O10 are resistant to acids but they are likely more sensitive than Cu members of the gillespite-group, since it has been argued that a key role in the acid resistance of MCuSi4O10 (M=Ca, Sr or Ba) is played by the high stability of Cu2+ in square planar coordination (Pabst 1959). However, the high stability of Cu2+in square planar coordination is not the only explanation for the acid resistance of MCuSi4O10. In fact, efenbergerite is much more stable than colinowensite even if both have Cu2+ in square planar coordination. Another key role should thus be played by the high stability of the network of SiO4 tetrahedra in the gillespite-group members. Nevertheless, EB pigment in harsh environments and on very long-time exposure (i.e., in the order of a few thousand years) has been reported to sufer from acid or salt-induced forms of decay, such as copper chloride cancer (see also Sect. 3.3.11). In the case of EB and other ancient Egyptian vitreous materials containing copper (e.g., Egyptian green and Egyptian faience), this kind of degradation seems due to the devitrifcation of the glass phase that is rich in elements, such as Na+ and K+ (Kakoulli 2009; Moussa et al. 2009; Moussa and Ali 2013). Devitrifcation likely occurs in archaeological EB even as a simple consequence of washout (Pradell et al. 2006).
It has also been observed in an archaeological octagonal stick made of CP (Doehne and Ma 2004) and arguably could happen also in CB. Even if cuprorivaite has antibacterial properties (Lamprecht et al. 1997; Tian et al. 2016) (see also Sect. 3.6), it has been suggested that a key role in the copper chloride cancer attack of EB could be played also by some fungal and bacterial species (Moussa et al. 2021). An attempt to artifcially induce copper chloride cancer on EB has been reported with prolonged exposure to concentrated HCl (Abadir 2014). It seems unclear if copper chloride cancer can afect directly cuprorivaite, efenbergerite, and wesselsite but may be worth noting that the use of strong acids such as HCl has been exploited to produce their artifcial exfoliation. However, in this case quite concentered HCl has been used in conjunction with an ultrasonic treatment that likely can greatly accelerate and amplify the process (Guo et al. 2015a, b, c). In this regard, it has been speculated that in the archaeological time scale, water-mediated delamination could take place even in archaeological samples of EB (Salguero et al. 2014; Orna and Fontani 2022). Indeed, some examples in the literature suggest the presence of nanoplatelets in various degrees of detachment in EB of archaeological sources (Canti and Heathcote 2002) and thus this possible pathway of decay should be taken into account by conservation scientists.
3.4.4 Stability in the alkaline environment
The stability of EB and CB in alkaline environments has been much less studied. However, it is possible to argue that they are very stable, since very well-preserved EB has been widely used in a highly alkaline environment (e.g., with slaked lime in fresco techniques or to paint on terracotta) both in the Early Middle Ages, in Roman times or even before (Riederer 1997; Coccato et al. 2017; Nicola et al. 2018a; Osanna and Rescigno 2022). At high temperatures, the presence of highly alkaline environment, e.g., due to soda or potash fuxes can decompose EB (Augusti 1967; Riederer 1997) and arguably CB, CP, and related 2D materials.
3.4.5 Efects of oxygen and light
Cuprorivaite, efenbergerite, wesselsite, and arguably also colinowensite are stable in oxidizing environments and are in general not afected by the presence of O2. Actually, O2 seems even necessary for the successful synthesis of EB, since it prevents the formation of Cu+ (Kiss et al. 2022).
Kendrick et al. point out that containing Cu2+, it is unlikely that those species show air instability but point out a possible light instability. In this regard, they reported a discoloration in some of their samples caused by irradiation with a high-intensity laser during their attempted Raman experiments. They ascribed it to the formation of a small amount of CuO that they reported also in X-ray difraction patterns acquired after exposing the samples to sunlight (Kendrick et al. 2007). This interesting hypothesis of Kendrick et al. would be consistent with the frst accounts for EB blackening (Lucas 1934; Scott 2016) but deserves further confrmation, since it contradicts the general consensus as reported by Riederer that EB exposed for thousands of years to the sun and heat on monuments in Egypt has overall retained its blue color (Riederer 1997). No instability under laser irradiation seems to have been reported by other scholars that performed wide Raman studies
(Baraldi et al. 2006) but it has been reported for high laser irradiation during upconversion experiments (Chen et al. 2015). Furthermore, it should be considered that explaining the blackening of archaeological EB with the formation of black CuO would also contradict the overall accepted conclusions of Lorna Lee (née Green) (Green 2001) and Daniels et al. (2004) who did not report signifcant amounts of CuO in samples of blackened EB. Godet et al. recently assessed the efects of synchrotron X-ray on EB and Egyptian green. Notably, Egyptian green was found to be more sensitive to X-rays than EB, turning brown at the lowest doses tested, while no color change occurred on EB at that X-ray intensity. Increasing the irradiation, three types of radiation-induced defects arise: E’, non-bonding oxygen hole, and aluminum hole centers (Godet et al. 2022).
3.5 Luminescence
3.5.1 NIR photoluminescence
All three Cu2+ members of the gillespite-group show a strong luminescence associated with the electronic transition 2 B2g— > 2 B1g with emission in the NIR I region at 900–1000 nm. The NIR luminescence of MCuSi4O10 can be triggered by the absorption of visible light in the red (overall between 600 and 630 nm) and, to a lesser extent, in the green region (Li et al. 2014). It can be induced also by absorptions in the NIR-I region at about 780 nm (King
Fig. 11 (Top) Refected Visible Light (VIS) of some blue pigments (bottom) Visible Induced Luminescence Imaging of the same pigments (colour fgure online). Adapted from Nicola and Nicola (2018)
et al. 2016) and in the UV (Binet et al. 2021). The three broadband absorptions in the VIS and NIR-I region are associated with parity-forbidden d–d transitions which are weakly allowed, despite approximate D4h symmetry at the copper center, due to vibronic coupling (Kendrick et al. 2007). The d–d transitions are, respectively, 2 B1g— > 2 A1g (~ 540 nm), 2 B1g— > 2 Eg (~ 630 nm), and 2 B1g— > 2 B2g (~ 780 nm) (Pozza et al. 2000; Kendrick et al. 2007; Accorsi et al. 2009; Verri 2009; Li et al. 2014; King et al. 2016) and are schematized in Fig. 6 in Sect. 3.3.1. Binet et al. report that the emission is excited much more efficiently by a factor of about 60 in the UV with an excitation peak at 5 eV (i.e., about 250 nm), corresponding to the valence band-conduction band transition (Binet et al. 2021). NIR excitation is noteworthy due to its higher depth of penetration. According to Borisov et al., λmax of emission of the NIR photoluminescence (PL) for the three MCuSi4O10 (M=Ca2+, Sr2+, and Ba2+) are, respectively, at 909 nm, 914 nm, and 948 nm (Borisov et al. 2013), while according to Cheng et al., λmax are at 932 nm, 936 nm, and 964 nm (Chen et al. 2015). Other authors report slightly different values with a general consensus on a red-shift in the Ca2+ to Ba2+ series (Pozza et al. 2000; Accorsi et al. 2009; Verri 2009; Li et al. 2014; King et al. 2016). The red-shift of the emission peak has been attributed to the increase of ionic radius from Ca2+ to Ba2+ which causes an expansion of the lattice resulting in a weaker crystal field (Pozza et al. 2000; Chen et al. 2015). The photoluminescence is based on the Cu2+ ion located on a fourfold axis, where, in square planar coordination, it links four SiO4 tetrahedra (Pozza et al. 2000; Kriss et al. 2016; Chiari 2018). It seems correlated also with the fact that despite their high concentration in the host matrix, Cu2+ ions are, indeed, weakly antiferromagnetically coupled and can be considered to show properties as they were independent Cu2+
Fig. 12 First three samples (from the left) are EB, CB, CP. The fourth is Egyptian green used as reference. a Regular photo (i.e., refected visible light); b Vis-induced NIR luminescence, under excitation from a red LED; The image was acquired in the 800–1000 nm range. In both images the grey scale contains a non-luminescent 99% refectance standard. Reproduced with permission from Verri (2009)
centers (Warner 2011; Barbar et al. 2017; Binet et al. 2021). Cu2+ centers, being isolated, release the excitation energy mainly via radiative decay and not through nonradiative pathways. In this regard, Zhuang and Tanabe pointed out how in Yb3+ doped crystals of cuprorivaite, i.e., Ca1−xCuSi4O10:Ybx, there seems to be an energy transfer mechanism that reduces the PL of Cu2+ centers. In fact, with the increase of the concentration of Yb, the PL intensity of Yb3+ increases too, reducing though the PL intensity of the Cu2+ due to the increased possibility of non-radiative charge transfer between the adjacent centers Cu2+ and Yb3+ (Zhuang and Tanabe 2012a, b). BaCuSi2O6 shows photoluminescence analogous to that of the three MCuSi4O10 (M=Ca, Sr, or Ba) (Pozza et al. 2000). According to Selvaggio et al. and Chen et al. its λmax of emission is centered at 924–925 nm (Chen et al. 2014a; Selvaggio et al. 2021), while according to Pozza et al., it is at 980 nm (Pozza et al. 2000). It may be worth noting that BaCu2Si2O7 (i.e., Chinese dark blue) does not seem to show any photo-induced luminesce in the visible and near-infrared spectral region (Chen et al. 2014b). Photoluminescence of EB, CB, and CP has been discovered at the end of the 1990s (Ajò et al. 1996; Pozza et al. 2000). Since 2009, it is used to identify the presence and map the distribution of the three pigments through multispectral imaging, i.e., the Visible Induced Luminescence imaging method, shortened as VIL (Verri 2008, 2009; Dyer et al. 2013). An exemplifying image of the NIR photoluminescence of EB obtained through VIL is reported in Fig. 11, while the comparison with CB, CP, and Egyptian green is displayed in Fig. 12. In the last decade, VIL has been widely used in Conservation Science and beyond, becoming an almost indispensable method in many archaeological sites and conservation institutions (Kriss et al. 2016). VIL method will be one of the foci of Part B of this review.
3.5.2 Lifetime of emission
Selvaggio and Kruss report that the lifetimes of emission of the NIR photoluminescence are generally in the range of 100–150 µs for EB, 60–100 µs for CB, and 6–30 µs for CP (Selvaggio and Kruss 2022). Other authors reported values slightly diferent but in the same order of magnitude (Borisov et al. 2013; Chen et al. 2014a; Berdahl et al. 2018). Fluorescence lifetimes are reduced by grinding (Berdahl et al. 2018; Linn et al. 2018), likely due to the introduction of defects by mechanical stress as typically observed also for other phosphors (Borisov et al. 2013; Berdahl et al. 2018). According to Selvaggio et al., the lifetimes of emission decrease signifcantly after exfoliation in nanosheets due to defects or changes in symmetry that either enable non-radiative decay pathways or increase the radiative rate constants. In particular, EB-nanosheets have been reported to show a fuorescence lifetime of 17 µs, CB-nanosheets of 8 µs, and CP-nanosheets of 7 µs (Selvaggio et al. 2021). The route of synthesis seems also to impact the lifetime of emission although a systematic study is needed to clearly understand how synthesis afects the emission lifetime (as well as quantum yield, see the following paragraph) (Linn et al. 2018). The luminescence decay of CP produced via hydrothermal synthesis has been reported to be 5.6 µs (Chen et al. 2014b). The study of luminescence lifetimes has been pioneered by Comelli et al. and Linn et al. to obtain useful data for archaeometric study (Comelli et al. 2016; Linn et al. 2018).
3.5.3 Quantum yield
In 2009 Accorsi et al. tested commercially available EB (i.e., Kremer Pigmente) for the quantum yield of the NIR photoluminescence, reporting an outstanding result of ΦEM =10.5% (Accorsi et al. 2009). The value is very high when compared with ΦEM of other NIR fuorophores which is often<2%. Furthermore, unlike EB, other NIR fuorophores often have low photostability and /or biocompatibility issues (Hong et al. 2017; Selvaggio et al. 2020; Selvaggio and Kruss 2022). The quantum yield of hydrothermally synthesized SrCuSi4O10 and BaCuSi4O10 has been reported to be, respectively, 8.5% and 6.9% (Chen et al. 2014a), while for BaCuSi2O6, a value of 0.9% has been measured. This latter lower level has been ascribed to the infuence of Cu–Cu interaction within the CuO4–CuO4 dimers in CP (Chen et al. 2014b). Features that have been reported to infuence the quantum yield (or at least the PL intensity) include the way of grinding (Sobik et al. 2021), the presence of CuO (Berdahl et al. 2018), and the route of synthesis (Berdahl et al. 2018; Nicola et al. 2019). Using a technique based on temperature measurements in full sunlight instead of the traditional de Mello method, Berdahl et al. measured an even much higher value of quantum yield for EB and wesselsite (Berdahl et al. 2018; Selvaggio and Kruss 2022). Being highly promising as a means to simplify the procedure of acquiring quantum yield data, this interesting and less laborious method seems to deserve further in-depth. The aim could be the confrmation of the results obtainable, crossing them with more traditional methods.
3.5.4 Thermoluminescence and cathodoluminescence
EB shows the rather rare feature of being a thermoluminescent material. It follows that it is directly datable with the thermoluminescence technique (Schvoerer et al. 1988). It seems, however, unclear if this feature is purely relative to cuprorivaite or if it is due to quartz grains present withing EB (see Sect. 3.1.1). So far, EB thermoluminescence has hardly been exploited. The cathodoluminescence of EB has been seldom investigated (Schvoerer et al. 1988; Binet et al. 2021; Yuryev et al. 2023). According to Kadikova et al. EB shows a characteristic peak at 874 nm when investigated through cathodoluminescence microspectroscopy. The technique is a promising method to identify pigments in cultural heritage (Kadikova et al. 2019; Yuryev et al. 2023).
3.5.5 Photothermal efect and photon up‑conversion
EB nanosheets and wesselsite nanosheets have been reported to exhibit an excellent photothermal efect by absorbing energy in the NIR-II window, a region of electromagnetic radiation that is generally not absorbed by biological tissue, i.e., characterized by low self-heating of the tissues, maximum permissible high exposure and depth of penetration (He et al. 2021; Yang et al. 2021a, b; Dong et al. 2022). The feature has been exploited in innovative medical devices (see also Sect. 3.6).
Furthermore, Chen et al. reported a bright and broadband photon up-conversion from NIR-I to visible in MCuSi4O10 (M=Ca, Sr, Ba). The efect is obtained via of-resonance pumping with a NIR laser diode and is mainly ascribed to the synergic efect of the laser-driven blackbody radiation and the peak broadening due to electron–phonon coupling. They use NIR-I wavelengths of 808 nm, 976 nm, and 1064 nm to produce red to bright white broadband light with the increase of laser power (Chen et al. 2015). Gao et al. report that it is possible to enhance photocatalytic nitrogen fxation arguably thanks to the up-conversion capability of CaCuSi4O10, which they report to convert NIR into visible
and UV light, thus improving the utilization efciency of the full solar spectrum. CaCuSi4O10 nanosheet that they used for this purpose are produced from EB obtained using as raw materials palygorskite, egg shells, and 5–15% of K2CO3 as a fux (Gao et al. 2022). No other studies have been so far performed about the up-conversion features of EB and related 2D materials. Confrmation of the results and a further indepth understanding of the mechanism seem promising opportunities for future research and innovative applications.
3.6 Biological efects and biocompatibility
3.6.1 General features
Many archaeological objects and papyri, e.g., the bust of Nefertiti, the Papyrus Berolina, and the Papyrus Ebers, have been observed to be less afected by biological decay in the parts painted with EB (Wiedemann et al. 1996; Lamprecht et al. 1997; Wiedemann and Berke 2001; Ramadan et al. 2019). The efects of EB, CB, and CP on the growth of lichens show that the three pigments, and especially EB, have a strong inhibitory efect on the growth of the algal component and to a minor extent of the fungal part (Lamprecht et al. 1997). CB has fungicidal properties similar to those of EB, while CP, being acid-sensitive, is purportedly less efective, since it can be decomposed by the oxalic acid produced by some microorganisms, e.g., lichens (Wiedemann and Berke 2001).
A strong antibacterial efect of cuprorivaite has been reported by Tian et al. using the bacteriological plate count method to test it against the proliferation of Gram-negative Escherichia coli (Tian et al. 2016). The anti-microbial efect of EB, CB, and CP has been related to the Cu2+ ions resulting from their slow solubilization. The efect is evident even if their solubility is very low, i.e., EB: 3. 10–7 mol. 1–1; CB:< 4. 10–8 mol. 1–1; CP:< 6. 10–8 mol. 1–1 (Lamprecht et al. 1997). The mechanisms besides the use of Cu2+ ions for the contrast of pathogenic microorganisms are well-known and have been treated by many authors (Thurman et al. 1989; Borkow and Gabbay 2009; Ramadan et al. 2019). In 2016, Tian et al., furthermore, report that Cu and Si ions from cuprorivaite have a synergistic efect on angiogenesis within a safe concentrations range. This can provide a better therapeutic activity and notably, it is free of cytotoxic efects for applications in the regeneration of human tissues (Tian et al. 2016).
The angiogenic efect and the safety of cuprorivaite have subsequently received growing interest for application in regenerative medicine (Zhang et al. 2020; Yang et al. 2021b; Dong et al. 2022). Tian et al. conclude that cuprorivaite has great potential for the preparation of wound healing dressings, but may not be suitable for bone repair applications, since it could inhibit osteogenesis (Tian et al. 2016). However, more recently, He et al. reported that 3D-printed scafolds coated with cuprorivaite nanosheets can be used to fght osteosarcoma achieving also enhanced bone tissue regeneration (He et al. 2021). Similar results have been reported also for wesselsite nanosheets (Yang et al. 2021a). The safety and biocompatibility of cuprorivaite nanosheets are also at the base of their use as fuorescent material in bioimaging and biophotonics (Selvaggio et al. 2020, 2021).
3.6.2 Historical awareness
From an archaeological point of view, it is possible that the angiogenetic and antibacterial efects of EB have been historically known and used. According to the description given by Pliny the Elder in 77 CE in Book XXXIII—57 of Naturalis Historia, EB could have been used to clean ulcers. Pliny states that “Caeruleum has the medicinal property of acting as a detergent upon ulcers. Hence, it is, that it is used as an ingredient in plasters, as also in cauteries.
However, it is very difcult to grind.” (vis in medicina ut purget ulcera; itaque et emplastris adiciunt, item causticis. Teritur autem difcillime) (Bostock and Riley 1857). Nevertheless, the passage is somehow unclear, since the Latin word caeruleum generally means EB, but sometimes it refers simply to a blue pigment (Riederer 1997). Since the immediately preceding sentence in the text of Pliny is relative to indigo, it is not possible to state conclusively that the Romans were aware of the angiogenetic efect of EB even if it is very likely since the features reported by Pliny, i.e., healing ulcers and being difcult to grind seems more appropriate for EB than for indigo. It cannot be ruled out that ancient Romans may have known the anti-algae properties of EB. Notably, in fact, between the frst century BCE and the frst century CE they used lumps of EB as mosaic tesserae that were almost exclusively used in environments rich in water, such as nymphaea and fountains (see also Fig. 15) (Boschetti 2011; Chiari 2018). It may be worth noting that some authors on the basis of a translation of documents of the 18th dynasty of Egypt, i.e., 1550–1292 BCE (Sethe 1961; Wiedemann and Bayer 1997; Wiedemann and Berke 2001) seem to have incorrectly inferred that EB could have been used in antiquity to preserve bread.
According to them, the production of “blue bread” would have been mentioned in the ancient text translated by Sethe (1961) and it could have denoted some knowledge about the conservation efect of EB that was possibly exploited to produce air-dried bread for emergency situations (Wiedemann and Bayer 1997; Wiedemann and Berke 2001). However, to a closer view, it seems that the text reported more specifcally “loaves” of EB with the term reliably referring to the shape of balls or blocks made of EB, such as those in which it was produced and which are sometimes found in archeological sites (see also Sect. 4.2). The fact that no other foods are described in the same inscription but other goods such as precious materials and stones is consistent with this interpretation as well as the fact that the presence of EB has never been reported in any of the quite numerous archaeological remains of bread from the Egyptian era analyzed so far (Glabau and Goldman 1938; Leek 1972; Samuel 2002).
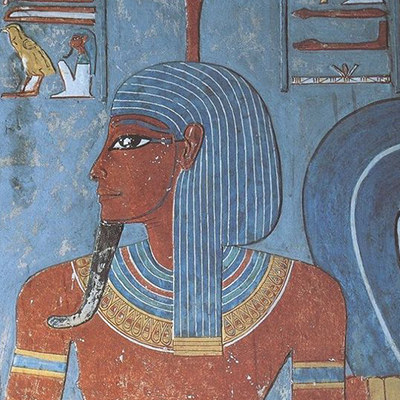
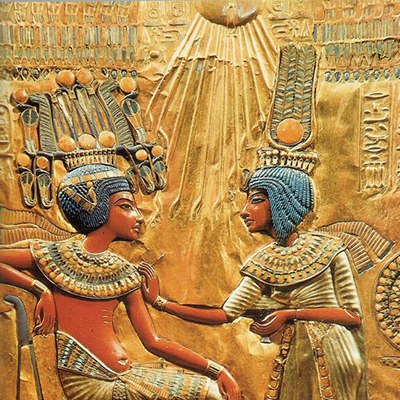
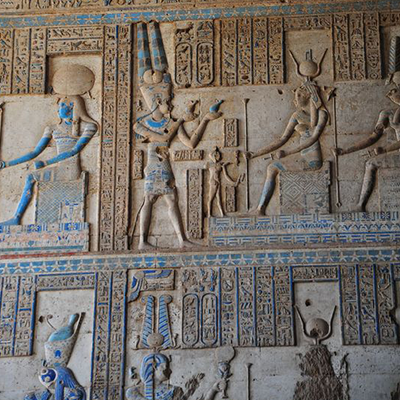
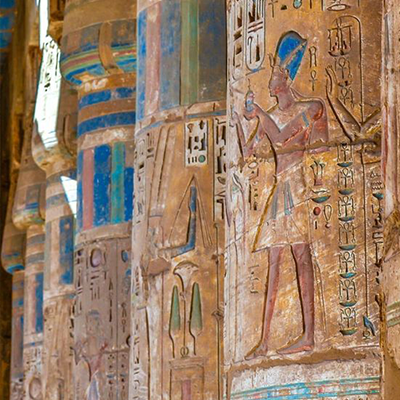
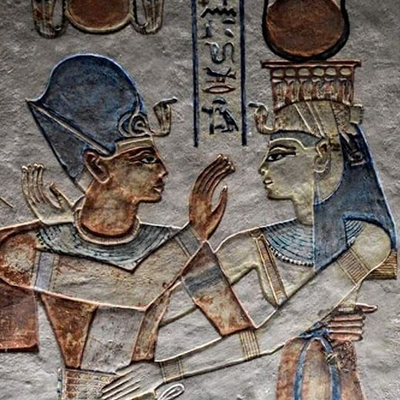
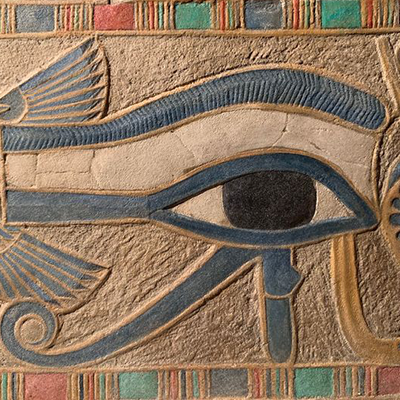